1. Introduction
Cholinergic-associated diseases are currently the major cause of neurological and neurodegenerative disabilities. Any cholinergic system dysfunction leads to various neurological diseases, such as Alzheimer Disease (AD) (
Abdel-Salam, 2011). Currently, AD is the most prevalent neurodegenerative disorder and the sixth most common cause of death in the United States (
Alzheimer's Association, 2015). The prevalence of the disease doubles every five years in people older than 65 years. This disease affects 30%–50% of all individuals by 85 years (
Querfurth & LaFerla, 2010). It is the most common type of dementia with loss of cell nucleus cholinergic base (
Alzheimer’s Disease International et al., 2015).
So far, there is no cure for AD. Current treatments are divided into using medications, psychotherapy, and rehabilitation cares. The amount of acetylcholine, as the mediating chemical mediator in memory, is reduced in AD patients. Injection of acetylcholinesterase inhibitors has increased the cholinergic function and temporarily improves the disease (
Borlongan, Sumaya & Moss, 2005;
Castro & Martinez, 2006). Although various drugs are used to increase the amount of acetylcholine in the nerve terminals, they are not considered as definitive treatment because these drugs are associated with gastrointestinal complications (
Schneider, 2000). Since the drugs are ineffective in treating and improving the suffered tissues, stem cell treatment is considered an effective strategy to recover the lost cells (
Borlongan, 2012). Among various types of stem cells, Mesenchymal Stem Cells (MSCs) are of particular interest in regenerative medicine with regard to their self-renewal properties for a relatively long time (
Chakari-Khiavi et al., 2019) and the ability to differentiate into multiple cell lineages, including osteoblasts (
Darzi et al., 2012), chondrocytes (
Khanmohammadi et al., 2012), adipocytes (
Atmani, Chappard & Basle, 2003) and myocytes (
Bana et al., 2017). They could also differentiate into various types of neural cell (
Azedi et al., 2014;
Azedi et al., 2017;
Neuhuber et al., 2004;
Yousefi, Sanooghi, Faghihi, Joghataei & Latifi, 2017;
Zemelko et al., 2013), such as oligodendrocytes (
Moayeri et al., 2017), motor neurons (
Darvishi, Tiraihi, Mesbah-Namin, Delshad & Taheri, 2017), and even cholinergic cells (
Marei et al., 2018).
The adipose tissue is distributed throughout the body and accounts for 15% to 20% of the body weight in men and 20% to 25% in women (
Bulfer & Eugene Allen, 1979). Human adipose tissue is considered a medical waste product and an excellent source of mesenchymal stem cells. They can be easily obtained from fat tissue with minimal side effects. Human Adipose tissue Derived from Mesenchymal Stem Cells (hAD-MSCs) can differentiate into adipogenic (
Gimble & Guilak, 2003), osteogenic (
El-Sayyad, Sobh, Khalifa & El-Sayyad, 2016), chondrogenic, and myogenic cells (
Wickham, Erickson, Gimble, Vail & Guilak, 2003). They also have immunogenic (
Kumar et al., 2012) and neuromuscular protecting properties (
Tajiri et al., 2014). There are also some reports indicating that mesenchymal stem cells derived from adipose tissue can differentiate into neural cells (
Choi, Cho, Seo, Yoon & Park, 2012;
Jang, Cho, Cho, Park & Jeong, 2010;
Marei et al., 2018;
Qu, Liu, Song, Li & Ge, 2013;
Radtke, Schmitz, Spies, Kocsis, & Vogt, 2009), such as motor neurons (
Darvishi et al., 2017;
Mohseni et al., 2019) and dopaminergic-like cells as well as cholinergic-like cells (
Marei et al., 2018).
In this regard, our study aimed to investigate the differentiation properties of hAD-MSCs into cholinergic-like cells in the presence of Retinoic Acid (RA) and Sonic hedgehog (Shh) as two morphogens responsible for rostral-caudal specification (
Wilson & Maden, 2005) of the neural tube, in vitro.
2. Methods
2.1. Isolation of human Adipose tissue Derived from Mesenchymal Stem Cells (hAD-MSCs)
The experiments were carried out according to the guidelines of the Ethics Committee of Iran University of Medical Sciences (Ethical code: IR.IUMS.REC1396.30694). After obtaining written consent from the healthy donors, fatty tissue was collected from a superficial layer of the abdomen during liposuction surgery of several individuals (age range: 25–46 years) at Motahari Hospital, Tehran City, Iran. The isolation of hAD-MSCs was performed according to Dubois et al. protocol (
Dubois et al., 2008). Before setting out the isolation process, fatty tissue was warmed in a 37°C water bath. All isolation steps were then performed under the hood in sterilized conditions. The fat tissue was transferred to a tube containing 1% penicillin/streptomycin (Invitrogen) dissolved in warm Phosphate-Buffered Saline (PBS, Invitrogen). The sample was washed with PBS several times to eliminate blood and connective tissue. Then the fatty tissue sample was divided into small parts using sterilized scissors and was transferred to a tube containing 0.1% collagenase type I (Gibco, 17100-017, USA) and 1% Bovine Serum Albumin (BSA) (dissolved in warm PBS, Invitrogen) for digestion. Afterward, the sample was kept in a water bath for 30 min with shaking intervals to ensure total digestion. After digesting the tissue, the tube was centrifuged at 1200 rpm for 5 min at room temperature. After discharging the supernatant, the pellet was suspended in 1% BSA/PBS solution and centrifuged at 1200 rpm. To remove red blood cells, the Red Blood Cell (RBC) lysis buffer was used according to the manufacturer׳s protocol. After centrifugation, the cell pellet was suspended in a medium containing DMEM/Ham’s F-12, 10% FBS, and 1% penicillin/streptomycin, then transferred to the tissue culture flasks (5000 cells/cm2) and kept in a humidified chamber (temperature: 37°C, CO2: 5 %, humidity: 98%). After forming colonies, the medium was exchanged with a fresh one every two days. At 70%-80% confluence, the cells were moved into three new flasks.
2.2. Characterization of hAD-MSCs
To characterize hAD-MSCs, the isolated cells at passage three were collected and incubated with fluorescent-conjugated monoclonal antibodies against CD90, CD73, CD45, CD44, CD29, CD105, and CD34 (all from BD Biosciences, USA) at 4°C for 40 min. The isotype-matched antibodies were used as control. The level of fluorescence greater than 95% of the one measured using the matched isotype control antibody was assumed as a positive expression. BD FACSCalibur flow cytometer (BD Biosciences, USA) was used to detect the expression of the antigens, and data analysis was performed by FlowJo software.
2.3. Induction of differentiation
Human AD-MSCs (1 × 105) were seeded in a 24-well plate containing the expansion medium and moved into a humidified chamber. Two days later, the medium was replaced with DMEM/F12, supplemented with 20% fetal bovine serum, 10 ng/mL basic fibroblast growth factor (FGF2, Sigma, USA), 2% B27 (Gibco, Germany), 250 mM isobutylmethylxanthine, and 100 mM β-mercaptoethanol for overnight. Afterward, the medium was changed with DMEM/F12, 0.2% B27 (Invitrogen, USA), 0.01 mM all-trans retinoic acid (RA; Sigma, USA), and 500 ng/mL Sonic hedgehog (Shh; R&D, USA). The medium was refreshed every 3 days. One week later, the cells were treated with DMEM/F12 and 0.2% B27 (Invitrogen, USA) for an additional 7 days. The medium was refreshed every 3 days.
2.4. Evaluation of differentiation
2.4.1. Immunophenotyping of differentiated hAD-MSCs
To quantify single properties of the differentiated cells, we evaluated the expression of Nestin, Islet-1, and Acetylcholinesterase (AChE) proteins by flow cytometry at 14 days post-induction. To do that, the treated cells were incubated with fluorescence conjugated antibodies against human Nestin (Chemi-Con, USA), Islet-1 (cat# SC30200, Santa Cruz, USA), and AChE (cat# 3739, Abcam, USA) at 4°C for 30 min. Then, an FACSCalibur flow cytometer (BD BioSciences) was used to detect expressions of these proteins. Positive expression was defined as the level of fluorescence greater than 95% of the one measured using the corresponding isotype-matched control antibodies. The results were analyzed using FlowJo software.
2.4.2. Cytofluorimetric analyses of the expression of neural markers in differentiated hAD-MSCs
After 14 days of differentiation, the cells were fixed in acetone at -20°C for 2 min. The fixed cells were blocked with 5% sheep serum for 10 min at room temperature and then incubated for 90 min at room temperature with human Nestin (1:300; Chemi-Con, USA), Islet-1 (cat# SC30200, Santa Cruz, USA), and AChE (cat# 3739, Abcam, USA). Subsequently, the cells were washed three times with 0.1% PBS/BSA and incubated with Fluorescein Isothiocyanate (FITC)-labeled sheep anti-rabbit IgG or sheep anti-mouse IgG at room temperature for 45 min in the dark. The nuclei were counterstained with 4',6-diamidino-2-phenylindole dihydrochloride at 1 μg/mL for 5 min. After washing, the coverslips were mounted in 70% PBS-glycerol and examined under a fluorescence microscope (Olympus, Tokyo, Japan).
2.4.3. Gene expression
Real-time PCR was carried out to determine the expression of the candid genes at 7 and 14 days of induction. Briefly, total cellular RNA was extracted using TRIzol reagent (Invitrogen, Germany). After digestion of DNA, the purity of RNA was quantified spectrometrically. Complementary DNA (cDNA) was synthesized using RevertAidTM H Minus First Strand cDNA Synthesis Kit (Fermentas, Canada). Real-time Polymerase Chain Reaction (PCR) reactions were carried out using the 7500 real-time PCR system (Applied Biosystems, USA). In each PCR reaction, ×1 SYBR Green PCR Master Mix (Applied Biosystems, USA) was mixed with 12 ng of cDNA and the related primers (
Table 1) in a total volume of 20 mL.
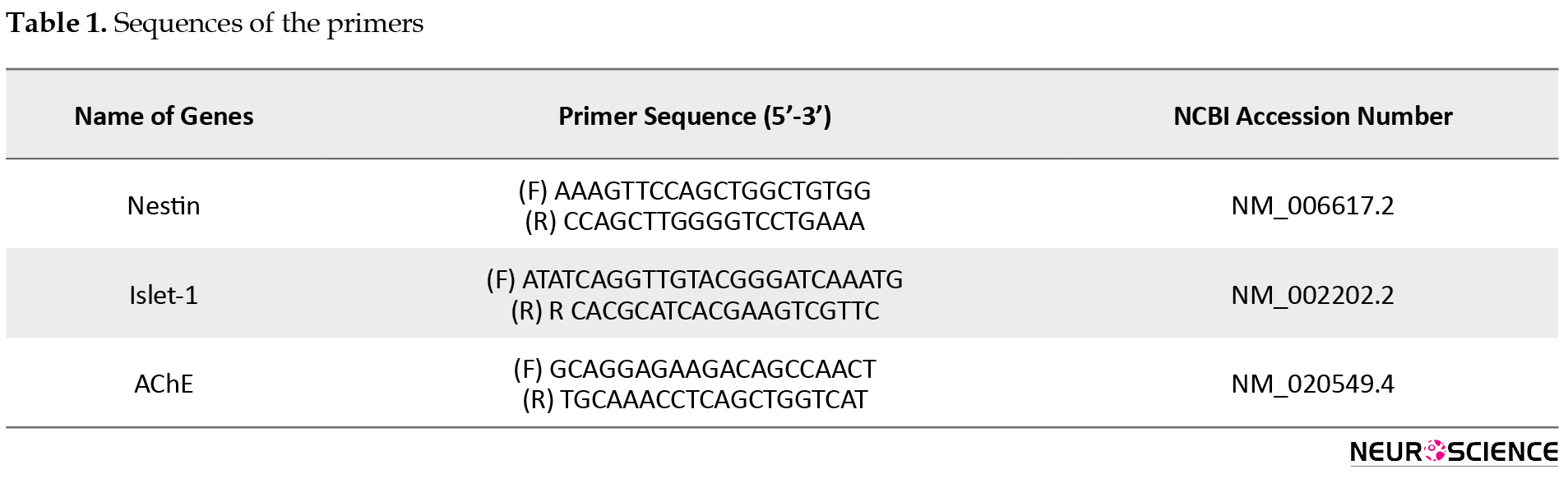
The expression of Glyceraldehyde 3-Phosphate Dehydrogenase (GAPDH) as an internal control was used to normalize the expression levels of the target genes in the treated cell groups compared with the same genes in hAD-MSCs at passage three.
2.5. Statistical analysis
The obtained data were presented as the Mean±Standard Error of Mean (SEM). The differences between study groups (n=3 in each group) were statistically assessed by nonparametric Mann-Whitney test in SPSS version 16. P values less than 0.05 were considered significant.
3. Results
3.1. Isolation and characterization of hAD-MSCs
The isolated cells could express CD105, CD29, CD90, CD73, and CD44, while they were negative for CD45, CD31, CD34, and HLADR markers (
Figure 1).
3.2. Evaluation of differentiation
3.2.1. Immunostaining
After induction of hAD-MSCs in a three-step protocol using RA and Shh, the cells could express neural and cholinergic antigens, including Nestin, AChE, and Islet-1, at 14 days post-induction (
Figure 2).
3.2.2. Cytofluorimetric analysis
Flow cytometry was used to evaluate the number of cells expressed cholinergic markers quantitatively. According to our data, mean±SD cell numbers of 17.78±3.86, 36.025±6.76, and 52.165±6.51 could express Nestin, AChE, and Islet-1, respectively (
Figure 3).
3.3. Gene expression
Gene expression analyses of hAD-MSCs treated with RA and Shh showed significant upregulation of candid genes, including Nestin as neural progenitor cell marker and Islet-1 and AChE as two cholinergic markers at 7 days post-induction when compared with non-treated MSCs at passage three as the control (P<0.05). The expression of the candid genes decreased one week later at 14 days post-induction, remarkably (
Figure 4).
4. Discussion
Loss of cholinergic neurons in AD has declined the quality of life in many patients worldwide. Several studies indicated the efficiency of stem cell therapy in the regeneration of cholinergic neurons. Nowadays, human AD-MSCs are considered a therapeutic agent in neurodegenerative disorders and one of the interesting cellular sources for stem cell therapies. These cells have valuable properties such as self-renewal, immunomodulatory characteristics, and differentiation potential into many lineages, particularly neural cells. To our knowledge, there are not enough reports about cholinergic differentiation of AD-MSCs using in AD treatment. Furthermore, improved protocols for MSCs induction will facilitate and ensure the reproducibility and standard production of MSCs for therapeutic applications in neurodegenerative diseases.
In our research, we demonstrated that isolated hAD-MSCs expressed typical levels of MSCs markers like CD105, CD29, CD90, CD73, and CD44. On the other hand, the absence of expression of CD45, CD31, CD34, and HLADR markers distinguished AD-MSCs from hematopoietic and endothelial cell types (
Amini et al., 2016).
To obtain cholinergic neurons from AD-MSCs, we implicated cholinergic differentiation by using RA and Shh. It is well established that RA has an essential role in neural differentiation (
Janesick, Wu & Blumberg, 2015). RA has been implicated in two aspects of spinal cord dorsoventral patterning. Firstly, in the induction of a subset of ventral interneurons (
Pierani, Brenner-Morton, Chiang & Jessell, 1999) and secondly, in the specification of motor neuron subtypes (
Sockanathan & Jessel, 1998). Also, Shh plays a critical role in the patterning of the vertebrate embryonic nervous system, including the brain and the spinal cord, during development. Moreover, Shh acts in a graded fashion to pattern the dorsal‐ventral axis of the vertebrate spinal cord (
Chiang et al., 1996).
Based on our results, hAD-MSCs could express neural and cholinergic neuron-specific markers, including Nestin, SMI-32, Islet-1, and AChE at the protein level in the presence of RA and Shh. SIM-32 is a nonphosphorylated neurofilament (NP-NF) marker and can serve as a relatively reliable marker of motor neurons (
Gotow & Tanaka, 1994). It is well established that loss of NP-NF such as SIM-32 is associated with AD (
Thangavel, Sahu, Van Hoesen & Zaheer, 2009). Therefore, increasing this marker in differentiated AD-MSCs can enhance their potential capacities in AD treatment.
Besides, we showed that differentiated AD-MSCs could express AChE, Nestin, and Islet-1 at the protein level.
Marei et al. (2018) also showed that using neural induction media containing RA for a total duration of 9 days and differentiated MSCs could express markers for immature and mature neurons (
Marei et al., 2018). AChE, the enzyme responsible for acetylcholine degradation to acetate and choline, has long been considered a marker of cholinergic neurons (
Zoli, 2000). When we evaluated the gene expression of neural and cholinergic neuron-specific markers in differentiated AD-MSCs, we observed that AChE, Nestin, and Islet-1 increased highly after 7 days; however, these values decreased one week later at 14 days. Increasing of islet-1 was reported by previous studies after neural induction by Shh and RA (
Darvishi et al., 2017;
Faghihi et al., 2016).
5. Conclusion
Overall, our results suggested that hAD-MSCs have a great capacity to differentiate into cholinergic-like cells. Human AD-MSCs have some advantages over other sources of MSCs, including simple extraction procedures, convenient cultivation, high abundance, high rate of proliferation, neuroprotective, and immunomodulatory effects (
Frese, Dijkman & Hoerstrup, 2016). Indeed, developing protocols for MSCs induction to cholinergic neuron needs more comprehensive studies. In this regard, more animal studies and applying functional techniques such as patch-clamp for better generalization are suggested.
Ethical Considerations
Compliance with ethical guidelines
This study was approved by the Ethics Committee of the Iran University of Medical Sciences (Code: IR.IUMS.REC.1396.30694).
Funding
This study was financially supported by the National Institute for Medical Research Development (NIMAD, Grant No. 958742) and Iran University of Medical Sciences (Grant No. 96-01-117-30694).
Authors' contributions
All authors equally contributed to preparing this article.
Conflict of interest
The authors declared no conflict of interest.
References
Abdel-Salam, O. M. E. (2011). Stem cell therapy for Alzheimer’s disease. CNS & Neurological Disorders - Drug Targets, 10(4), 459-85. [DOI:10.2174/187152711795563976] [PMID]
Amini, N., Vousooghi, N., Hadjighassem, M., Bakhtiyari, M., Mousavi, N., & Safakheil, H., et al. (2016). Efficacy of human adipose tissue-derived stem cells on neonatal bilirubin encephalopathy in rats. Neurotoxicity Research, 29(4), 514-24. [DOI:10.1007/s12640-016-9599-3] [PMID]
Alzheimer's Association. (2015). 2015 Alzheimer's disease facts and figures. Alzheimer's & Dementia, 11(3), 332-84. [DOI:10.1016/j.jalz.2015.02.003] [PMID]
Alzheimer’s Disease International, Wimo, A., Ali, G. C., Guerchet, M., Prince, M., & Prina, M., et al. (2015). World Alzheimer report 2015. The global impact of dementia: An analysis of prevalence, incidence, cost and trends. Retrieved from https://www.alzint.org/resource/world-alzheimer-report-2015/
Atmani, H., Chappard, D., & Basle, M. F. (2003). Proliferation and differentiation of osteoblasts and adipocytes in rat bone marrow stromal cell cultures: Effects of dexamethasone and calcitriol. Journal of Cellular Biochemistry, 89(2), 364-72. [DOI:10.1002/jcb.10507] [PMID]
Azedi, F., Kazemnejad, S., Zarnani, A. H., Behzadi, G., Vasei, M., & Khanmohammadi, M., et al. (2014). Differentiation potential of menstrual blood- versus bone marrow-stem cells into glial-like cells. Cell Biology International, 38(5), 615-24. [DOI:10.1002/cbin.10245] [PMID]
Azedi, F., Kazemnejad, S., Zarnani, A. H., Soleimani, M., Shojaei, A., & Arasteh, Sh. (2017). Comparative capability of menstrual blood versus bone marrow derived stem cells in neural differentiation. Molecular Biology Reports, 44(1), 169-82. [DOI:10.1007/s11033-016-4095-7] [PMID]
Bana, N., Sanooghi, D., Soleimani, M., Hayati Roodbari, N., Alavi Moghaddam, S., & Joghataei, M. T., et al. (2017). A comparative study to evaluate myogenic differentiation potential of human chorion versus umbilical cord blood-derived mesenchymal stem cells. Tissue and Cell, 49(4), 495-502. [DOI:10.1016/j.tice.2017.05.003] [PMID]
Borlongan, C. V., Sumaya, I. C., & Moss, D. E. (2005). Methanesulfonyl fluoride, an acetylcholinesterase inhibitor, attenuates simple learning and memory deficits in ischemic rats. Brain Research, 1038(1), 50-8. [DOI:10.1016/j.brainres.2005.01.028] [PMID]
Borlongan, C. V. (2012). Recent preclinical evidence advancing cell therapy for Alzheimer’s disease. Experimental Neurology, 237(1), 142-6. [DOI:10.1016/j.expneurol.2012.06.024] [PMID] [PMCID]
Bulfer, J., & Eugene Allen, C. (1979). Fat cells and obesity. BioScience, 29(12), 736-41. [DOI:10.2307/1307668]
Castro, A., & Martinez, A. (2006). Targeting beta-amyloid pathogenesis through acetylcholinesterase inhibitors. Current Pharmaceutical Design, 12(33), 4377-87. [DOI:10.2174/138161206778792985] [PMID]
Chakari-Khiavi, F., Dolati, S., Chakari-Khiavi, A., Abbaszadeh, H., Aghebati-Maleki, L., & Pourlak, T., et al. (2019). Prospects for the application of mesenchymal stem cells in Alzheimer’s disease treatment. Life Sciences, 231, 116564. [DOI: 10.1016/j.lfs.2019.116564] [PMID]
Chiang, Ch., Litingtung, Y., Lee, E., Young, K. E., Corden, J. L., & Westphal, H., et al. (1996). Cyclopia and defective axial patterning in mice lacking Sonic hedgehog gene function. Nature, 383(6599), 407-13. [DOI:10.1038/383407a0] [PMID]
Choi, Y. K., Cho, H., Seo, Y. K., Yoon, H. H., & Park, J. K. (2012). Stimulation of sub-sonic vibration promotes the differentiation of adipose tissue-derived mesenchymal stem cells into neural cells. Life Sciences, 91(9-10), 329-37. [DOI:10.1016/j.lfs.2012.07.022] [PMID]
Darvishi, M., Tiraihi, T., Mesbah-Namin, S. A., Delshad, A. R., & Taheri, T. (2017). Motor neuron transdifferentiation of neural stem cell from adipose-derived stem cell characterized by differential gene expression. Cellular and Molecular Neurobiology, 37(2), 275-89. [DOI:10.1007/s10571-016-0368-x] [PMID]
Darzi, S., Zarnani, A. H., Jeddi-Tehrani, M., Entezami, K., Mirzadegan, E., & Akhondi, M. M., et al. (2012). Osteogenic differentiation of stem cells derived from menstrual blood versus bone marrow in the presence of human platelet releasate. Tissue Engineering Part A, 18(15-16), 1720-8. [DOI:10.1089/ten.TEA.2011.0386] [PMID] [PMCID]
Dubois, S., Floyd, E. Z., Zvonic, S., Kilroy, G., Wu, X., & Carling, S., et al. (2008). Isolation of human adipose-derived stem cells from biopsies and liposuction specimens. In D. J. Prockop, B. A. Bunnell, & D. G. Phinney (Eds.), Mesenchymal stem cells. Methods in molecular biology™ (pp. 69-79). Vol. 449. Totowa, NJ: Humana Press. [DOI:10.1007/978-1-60327-169-1_5] [PMID]
El-Sayyad, H. I. H., Sobh, M. A., Khalifa, S. A., & El-Sayyad, O. K. R. A. (2016). Adipose derived mesenchymal stem cell differentiation into adipogenic and osteogenic stem cells. Studies on Stem Cells Research and Therapy, 2(1), 017-24. [DOI:10.17352/sscrt.000008]
Faghihi, F., Mirzaei, E., Ai, J., Lotfi, A., Sayahpour, F. A., & Ebrahimi Barough, S., et al. (2016). Differentiation potential of human chorion-derived mesenchymal stem cells into motor neuron-like cells in two- and three-dimensional culture systems. Molecular Neurobiology, 53(3), 1862-72. [DOI:10.1007/s12035-015-9129-y]
Frese, L., Dijkman, P. E., & Hoerstrup, S. P. (2016). Adipose tissue-derived stem cells in regenerative medicine. Transfusion Medicine and Hemotherapy, 43(4), 268-74. [DOI:10.1159/000448180] [PMID] [PMCID]
Gimble, J. M., & Guilak, F. (2003). Adipose-derived adult stem cells: Isolation, characterization, and differentiation potential. Cytotherapy, 5(5), 362-9. [DOI:10.1080/14653240310003026] [PMID]
Gotow, T., & Tanaka, J. (1994). Phosphorylation of neurofilament H subunit as related to arrangement of neurofilaments. Journal of Neuroscience Research, 37(6), 691-713. [DOI:10.1002/jnr.490370604] [PMID]
Janesick, A., Wu, S. C., & Blumberg, B. (2015). Retinoic acid signaling and neuronal differentiation. Cellular and Molecular Life Sciences, 72(8), 1559-76. [DOI:10.1007/s00018-014-1815-9] [PMID]
Jang, S., Cho, H. H., Cho, Y. B., Park, J. S., & Jeong, H. S. (2010). Functional neural differentiation of human adipose tissue-derived stem cells using bFGF and forskolin. BMC Cell Biology, 11, 25. [DOI:10.1186/1471-2121-11-25] [PMID] [PMCID]
Khanmohammadi, M., Khanjani, S., Sani Bakhtyari, M., Zarnani, A. H., Edalatkhah, H., & Akhondi, M. M., et al. (2012). Proliferation and chondrogenic differentiation potential of menstrual blood- and bone marrow-derived stem cells in two-dimensional culture. International Journal of Hematology, 95(5), 484-93. [DOI:10.1007/s12185-012-1067-0] [PMID]
Kumar, G., Hara, H., Long, C., Shaikh, H., Ayares, D., & Cooper, D. K. C., et al. (2012). Adipose-derived mesenchymal stromal cells from genetically modified pigs: Immunogenicity and immune modulatory properties. Cytotherapy, 14(4), 494-504. [DOI:10.3109/14653249.2011.651529] [PMID] [PMCID]
Marei, H. E. S., El-Gamal, A., Althani, A., Afifi, N., Abd-Elmaksoud, A., & Farag, A., et al. (2018). Cholinergic and dopaminergic neuronal differentiation of human adipose tissue derived mesenchymal stem cells. Journal of Cellular Physiology, 233(2), 936-45. [DOI:10.1002/jcp.25937] [PMID]
Moayeri, A., Nazm Bojnordi, M., Haratizadeh, S., Esmaeilnejad-Moghadam, A., Alizadeh, R., & Ghasemi Hamidabadi, H. (2017). Transdifferentiation of human dental pulp stem cells into oligoprogenitor cells. Basic and Clinical Neuroscience, 8(5), 387-94. [DOI:10.18869/nirp.bcn.8.5.387] [PMID] [PMCID]
Mohseni, R., Ashrafi, M. R., Ai, J., Nikougoftar, M., Mohammadi, M., & Ghahvechi-Akbari, M., et al. (2019). Overexpression of SMN2 gene in motoneuron-like cells differentiated from adipose-derived mesenchymal stem cells by ponasterone A. Journal of Molecular Neuroscience, 67(2), 247-57. [DOI:10.1007/s12031-018-1232-x] [PMID]
Neuhuber, B., Gallo, G., Howard, L., Kostura, L., Mackay, A., & Fischer, I. (2004). Reevaluation of in vitro differentiation protocols for bone marrow stromal cells: Disruption o f actin cytoskeleton induces rapid morphological changes and mimics neuronal phenotype. Journal of Neuroscience Research, 77(2), 192-204. [DOI:10.1002/jnr.20147] [PMID]
Pierani, A., Brenner-Morton, S., Chiang, Ch., & Jessell, T. M. (1999). A sonic hedgehog-independent, retinoid-activated pathway of neurogenesis in the ventral spinal cord. Cell, 97(7), 903-15. [DOI:10.1016/S0092-8674(00)80802-8]
Qu, X., Liu, T., Song, K., Li, X., & Ge, D. (2013). Differentiation of reprogrammed human adipose mesenchymal stem cells toward neural cells with defined transcription factors. Biochemical and Biophysical Research Communications, 439(4), 552-8. [DOI:10.1016/j.bbrc.2013.09.005] [PMID]
Querfurth, H. W., & LaFerla, F. M. (2010). Alzheimer’s disease. The New England Journal of Medicine, 362(4), 329-44. [DOI:10.1056/NEJMra0909142] [PMID]
Radtke , C., Schmitz, B., Spies, M., Kocsis, J. D., & Vogt, P. M. (2009). Peripheral glial cell differentiation from neurospheres derived from adipose mesenchymal stem cells. International Journal of Developmental Neuroscience, 27(8), 817-23. [DOI:10.1016/j.ijdevneu.2009.08.006] [PMID]
Schneider, L. S. (2000). A critical review of cholinesterase inhibitors as a treatment modality in Alzheimer’s disease. Dialogues in Clinical Neuroscience, 2(2), 111-28. [DOI:10.31887/DCNS.2000.2.2/lschneider] [PMID] [PMCID]
Sockanathan, Sh., & Jessel, T. M. (1998). Motor neuron-derived retinoid signaling specifies the subtype identity of spinal motor neurons. Cell, 94(4), 503-14. [DOI:10.1016/S0092-8674(00)81591-3]
Tajiri, N., Acosta, S. A., Shahaduzzaman, M., Ishikawa, H., Shinozuka, K., & Pabon, M., et al. (2014). Intravenous transplants of human adipose-derived stem cell protect the brain from traumatic brain injury-induced neurodegeneration and motor and cognitive impairments: Cell graft biodistribution and soluble factors in young and aged rats. Journal of Neuroscience, 34(1), 313-26. [DOI:10.1523/JNEUROSCI.2425-13.2014] [PMID] [PMCID]
Thangavel, R., Sahu, S. K., Van Hoesen, G. W., & Zaheer, A. (2009). Loss of nonphosphorylated neurofilament immunoreactivity in temporal cortical areas in Alzheimer’s disease. Neuroscience, 160(2), 427-33. [DOI:10.1016/j.neuroscience.2009.02.037] [PMID] [PMCID]
Wickham, M. Q., Erickson, G. R., Gimble, J. M., Vail, T. P., & Guilak, F. (2003). Multipotent stromal cells derived from the infrapatellar fat pad of the knee. Clinical Orthopaedics and Related Research, 412, 196-212. [DOI:10.1097/01.blo.0000072467.53786.ca] [PMID]
Wilson, L., & Maden, M. (2005). The mechanisms of dorsoventral patterning in the vertebrate neural tube. Developmental Biology, 282(1), 1-13. [DOI:10.1016/j.ydbio.2005.02.027] [PMID]
Yousefi, B., Sanooghi, D., Faghihi, F., Joghataei, M. T., & Latifi, N. (2017). Evaluation of motor neuron differentiation potential of human umbilical cord blood- derived mesenchymal stem cells, in vitro. Journal of Chemical Neuroanatomy, 81, 18-26. [DOI:10.1016/j.jchemneu.2017.01.003] [PMID]
Zemelko, V. I., Kozhukharova, I. B., Alekseenko, L. L., Domnina, A. P., Reshetnikova, G. F., & Puzanov, M. V., et al. (2013). Neurogenic potential of human mesenchymal stem cells isolated from bone marrow, adipose tissue and endometrium: A comparative study. Cell and Tissue Biology, 7(3), 235-44. [DOI:10.1134/S1990519X13030140]
Zoli, M. (2000). Distribution of cholinergic neurons in the mammalian brain with special reference to their relationship with neuronal nicotinic acetylcholine receptors. In F. Clementi, D. Fornasari, & C. Gotti (Eds.), Neuronal nicotinic receptors. Handbook of experimental pharmacology (pp. 13-30). Vol. 144. Berlin/Heidelberg: Springer. [DOI:10.1007/978-3-642-57079-7_2]