1. Introduction
Pesticides have became a vital tool in agriculture systems for plant protection against pests and diseases during the last century, allowing for a noticeable rise in the volume of crop production by eradicating unwanted insects. Nevertheless, excessive exposure to these compounds can lead to neurological and cognitive disorders (Doherty, 2006; Manjeet & Charles, 2012; Yan, Zhang, Liu, & Yan, 2016). These compounds, while being in contact with the human body, induce neurotoxic alterations associated with oxidative stress and histological brain damage. Carbamates are commonly used in home gardens and on farms as insecticides, fungicides, nematocides, molluscicides, and acaricides.
Methomyl (MET) is a carbamate insecticide introduced by DuPont in 1966 with anticholinesterase activity, which has been used to control harmful insects in the agriculture and public health programs. However, contamination of the environment with MET might pose serious harm to nontarget organisms. The human can be exposed to MET pesticides through contact with the skin, ingestion, or inhalation. MET was classified as a highly hazardous (class 1B) compound by the World Health Organisation (WHO, 2004).
Many studies have reported that pesticide is a strong toxicant that can cause central nervous system disorders (Andersen, Debes, Wohlfahrt-Veje Murata, Grandjean, 2015; Levin et al., 2002). The hippocampus is particularly vulnerable to the risks posed by pesticides resulting in behavioral and cognitive dysfunctions, such as memory disorders (Chen et al., 2012; Virginia et al., 2011). Several studies have shown that exposure to Acetylcholinesterase (AChE) inhibitor pesticides, such as carbamate and organophosphorus can induce learning and memory impairments (Olga, Timofeeva, Roeggeb, Seidlerc, & Slotkinac, 2008; Karczmar, 1998), and also repeated exposure to pesticides, affects hippocampal-dependent spatial learning and memory (Parihar, Hattiangady, Shuai, & Shetty, 2013; Nasuti et al., 2014; Terry et al., 2003).
For many pesticides, histopathological alterations in the brain and induction of oxidative stress are one of the major mechanisms of their action (Qing et al., 2010; El-Demerdash, 2011). Some studies have suggested that oxidative stress can have significant implications in the neuropathology induced by pesticide exposure. The brain is especially vulnerable to oxidative damage due to its high content of lipids and high oxygen consumption, which can exacerbate oxidative stress. During recent decades, the antioxidants have been shown to be important for the protection against oxidative damage induced by pesticide exposure. Antioxidants are classified as follows: exogenous (natural or synthetic), such as vitamin C, polyphenols, and carotenoids and endogens compounds that both play an important role in the free radical scavenging activity and elimination of the Reactive Oxygen Species (ROS). Herbal essential oils present excellent antioxidant activities and are used to cure several diseases (Kang, Na, & Kim, 2010; Valiollah, Alireza, & Hadi, 2004). Pelargonium graveolens (P. graveolens) commonly called a rose-scented geranium is a medicinal herb. This plant has the highest antioxidant activity. Geranium Essential Oil (EO) is used to treat different disorders, including inflammation, dysentery, heavy menstrual flow hemorrhoids, cancer, and reproductive system disease (Boukhris, Bouaziz, Feki, El Feki, Sayadi, 2012; Ben Slima, Ali, & Barkallah, 2013).
To our knowledge, the potential protective effect of P. graveolens EO on MET-induced Spatial Working Memory (SWM) deficits, oxidative stress, and histological alterations in the CA1 region of the hippocampus has not yet been explored. Based on the above information, we hypothesized that P. graveolens EO can reduce hippocampal damage induced by MET.
The aims of this study were as follows: (i) evaluating the effect of MET exposure on SWM using the Y-maze; (ii) assessing the effect of MET on lipid peroxidation, antioxidant defense system, and histopathological changes in the CA1 region of the hippocampus; and (iii) investigating the possible protective role of P. graveolens EO against MET insecticide.
2. Materials & Methods
2.1. Chemicals
MET (S-methyl N-(methylcarbamoyloxy) is a carbamate insecticide with a molecular formula of C5 H10N2O2S (CAS registry number: 16752-77-5). A commercial formulation of the MET (Lannate® 25% WP) containing 25% of active ingredient was purchased from the local market and used in the experiments. Other chemical products used in this study were purchased from the Sigma Chemical Co. (St Louis, France).
2.2. Essential oil
The EO of P. graveolens was obtained from the Laboratory of Ethnobotany and Natural Substances, ENS Kouba, Algiers, Algeria. The specimen of P. graveolens EO has been kept in our laboratory for future reference. The ten most important components of P. graveolens EO are listed in Table 1 with their retention index and percentage composition.
.png)
2.3. Animals
Experiments were carried out on 24 adult male Wistar rats (weighing 150-250 g; aged 6 to 10 weeks) obtained from the Pasteur Institute of Kouba (Algiers, Algeria). The rats were housed in polypropylene cages and food and water were available ad libitum throughout the study. They were maintained under laboratory conditions as follows: temperature: 24±2 °C, light cycle (12 light/12 dark), relative humidity: 40 – 60%. The animals were used and treated in accordance with the Institutional Guidelines for Animal Care, and the protocol was approved by the Local Ethics Committee.
2.4. Experimental design
After an acclimatization period of two weeks, animals were randomly divided into four groups of six each and they treated as follows: group I received only the vehicle Tween 20 solution (0.9% NaCl containing 0.1% of Tween 20); group II received MET at a dose of 2 mg/kg b.w (equivalent to 1/8 of DL50 MET; Djeffal et al. (2015)); group III received only EO of P. graveolens at a dose of 75 mg/kg b.w (diluted in a 1% solution of Tween 20); group IV received MET and EO of P. graveolens after 90 min of MET administration. Rats were administered their respective doses orally by gavage, every day for 28 consecutive days and adjusted weekly for body weight changes. The selective dose of P. graveolens EO (75 mg/kg b.w) as described by Boukhris, Bouaziz, Feki, El Feki and Sayadi (2012).
2.5. Assessing SWM by measuring spontaneous alternation
2.5.1. Apparatus
It is well known that spontaneous alternation is a measure of SWM, short-term memory, general locomotor activity, and stereotypic behavior (Swonger & Rech, 1972; Deacon & Rawlins, 2006). Spontaneous alternation was assessed using a Y-maze composed of three equally spaced arms (120°, 65 cm long, 15 cm width, and 31cm high). The test is based on the ability of animals to enter an arm of the Y-maze not entered in the previous choices.
2.5.2. Experimental procedure
SWM performance was assessed on the day before the first treatment (day 1) and day 28 after the last treatment, by recording spontaneous alternation behavior in a Y-maze. Behavior was conducted in a large quiet room between 10 a.m. and 11 a.m. The rat was placed in the maze and allowed to freely explore for 5 min. The series of arm entries, including returns into the same arm, were visually recorded. An alternation was defined as entries into all three arms on consecutive occasions. The number of maximum alternations was considered as the total number of arm entries minus two, and the percentage of alternation was calculated as (actual alternations/maximum alternations)×100. The apparatus was cleaned with 5% alcohol and allowed to dry between sessions.
2.6. Determination of the enzyme activity and oxidative stress markers
2.6.1. Preparation of the tissue homogenates
The rats were euthanized under ether anesthesia and the brain quickly removed and the fresh hippocampus was dissected and homogenized (1/10 [w/v]) in cold physiological saline (0.9% [w/v] NaCl) using a Polytron homogenizer (T25 UtraTurax; IKAWerke, Staufen, Germany). Homogenates were then centrifuged at 10,000 x g for 20 min at 4 °C and the resultant supernatant was then divided into Eppendorf tubes and stored at –20 °C until being used for the determination of enzyme activities and oxidative stress markers. Protein concentration was estimated by the procedure of Bradford (1976), using bovine serum albumin as a standard.
2.6.2. Enzyme assays
The transaminase enzymes, including aspartate aminotransferase (AST) and alanine aminotransferase (ALT) activities in the hippocampal region were measured spectrophotometrically according to the method by Bergmeyer et al. (1978). Alkaline phosphatase (ALP) activity in the hippocampus was measured according to the method by Bowers and McComb (1975). AST, ALT, and ALP enzyme activities are expressed as U/mg protein. The Acetylcholinesterase (AChE) activity in the hippocampus was estimated by the method of Ellman (1959). Briefly, in 1,000 μl of PBS (0.1 M, pH: 7.4), we added 50 μl of 5-5´-dithiobis (2-nitrobenzoic acid), 50 μl of acetylcholine iodide substrate, and 50 μl of supernatant. Absorbance values were measured every 30 s for 3 min at room temperature (24 °C). The complex was measured at 412 nm against the blank solution. The data units were displayed in μmol/min/mg protein.
2.6.3. Oxidative stress parameters
2.6.3.1. Estimation of Lipid Peroxidation (LPO) level
The LPO levels in the hippocampus homogenates are determined based on the Buege and Aust (1984) method. Briefly, 0.5 ml of supernatant was mixed with 1 ml of Trichloroacetic Acid (TCA) to precipitate proteins, and then was solved and centrifuged at 2,500 x g for 10 min at 4°C. The supernatant was mixed with Hydrochloric acid (HCl) (0.6 M) and Thiobarbituric Acid (TBA) was dissolved in Tris, and the mixture was then heated at 80 °C for 10 min and cooled. The absorbance of the TBA-Malondialdehyde (MDA) complex was measured at 530 nm and LPO was expressed as TBARS (nmol MDA/mg protein).
2.6.3.2. Determination of the reduced Glutathione (GSH) level
Reduced GSH level in the hippocampus was estimated by the method of Ellman (1959) modified by Jollow et al. (1974). Briefly, 0.8 ml of the homogenate hippocampus was added to 0.3 ml of 0.25% sulfosalicylic acid for deproteinization, the mixture was incubated for 1 hour at 4°C, and then the tubes were centrifuged at 2000g for 15 min. Next, 0.5 ml of the supernatant was taken and mixed with 0.025 ml of Ellman’s reagent (DTNB 0.01 M) and 1 ml phosphate buffer (0.1 M, pH: 7.4). The absorbance was recorded at 412 nm after 10 min. Total GSH content was expressed as nmol/mg of protein.
2.6.3.3. Evaluation of antioxidant enzyme activities
The Activity of Catalase (CAT): The activity of CAT in the hippocampus homogenate was estimated using the method by Aebi (1984). The enzyme catalyzes the decomposition of hydrogen peroxides, and the level of enzyme activity was followed by monitoring the absorption at 240 nm. One unit of CAT activity is defined as the number of enzymes required to decompose 1 mmol of hydrogen peroxide (H2O2) in 1 min. The enzyme activity was calculated as μmol H2O2 consumed/min/mg protein.
The activity of Glutathione Peroxidase (GPx): The activity of GPx in the hippocampus was determined by the method of Flohe and Gunzler (1984). Briefly, the assay mixture consisted of 0.3 ml phosphate buffer (0.1M, pH: 7.4), 0.2 ml reduced GSH (2 mM), 1mM of H2O2, 0.1ml of sodium azide (10 mM), and 0.3 ml of hippocampus homogenate. The mixture was incubated for 15 min at 37 °C, and the reaction was finished by the addition of 0.5 ml of 5% TCA. Tubes were centrifuged at 2000g for 15 min, and the supernatant was collected. Totally, 0.2 ml phosphate buffer (0.1M, pH: 7.4) and 0.7 ml of 5,5ʹ-dithio-bis-(2-nitrobenzoic acid) [DTNB (0.4 mg/mL)] were added to reaction supernatant. After mixing, absorbance was measured at 420 nm. GPx activity was expressed as nmol GSH/mg protein.
The activity of the Glutathione-S-Transferase (GST): The activity of GST was assessed according to the method provided by Habig, Pabst and Jakoby (1974) using Chloro-Dinitro Benzene (CDNB) as the substrate. The absorbance was measured spectrophotometrically at 340 nm at the 30s of intervals for 3 min. GST activity was expressed as nmol CDNB/min/mg protein.
2.7. Histopathology study
The brain was removed from the cranial cavity, immediately fixed in 10% formal saline solution for 48 h, dehydrated in increasing concentrations of alcohol, and embedded in paraffin. The brain was then cut into 5-μm thick coronal slices. After staining with cresyl violet (Nissl), sections of Corn Ammon 1 (CA1) region of the hippocampus were examined under light microscopy (Max300: Premiere®) with an attached digital camera (MA89: Premiere®, 5.0 megapixels with 2592 x 1944 resolution) using Cam2V5.4 software for determination of the pathological changes.
2.8. Statistical Analysis
All numerical data are expressed as Mean±Standard Error of Measurement (SEM). A comparison between the two means was carried out using a One-way Analysis of Variance (ANOVA). Statistical analysis was done using SPSS (version 14.0, Chicago, IL). Differences were considered significant at P<0.05.
3. Results
3.1. Effect of the treatment on SWM
Figure 1 shows the percentage of spontaneous alternation behavior (as an index of SWM) and the total number of arm entries on days 1 and 28 after the last treatment. In Figure A, the percentage of spontaneous alternation was significantly lower (P<0.01) in MET-treated rats compared with the control group on day 28 (Figure 1A). On the other hand, the exploration rates were not affected by MET exposure as the total number of arm entries; there was no statistically significant difference in MET-treated rats compared with the controls (Figure 1B). Cotreatment of P. graveolens EO to the MET-treated group significantly improved SWM performance in comparison with the MET group (P<0.05) (Figure 1A).
.png)
3.2. Effect of MET treatment on enzyme activities in the hippocampus
Exposure to MET caused a significant increase in the enzyme activities of AST, ALT, and ALP in the hippocampus tissue compared with controls (P<0.001, P<0.01, P<0.01, respectively), indicating the induction of severe neurotoxicity (Table 2). However, a significant decrease in AChE activity in MET-treated rats was observed compared with the control group (P<0.001). A decrease in AChE activity indicated the inhibition of its activity by MET pesticide. The coadministration of P. graveolens EO prevented the activities of the above-mentioned enzymes in the hippocampus.
.png)
3.3. Effect of MET on markers of oxidative stress in hippocampus tissue
3.3.1. Lipid peroxidation levels
Treatment with MET caused a significant increase in the levels of LPO (Figure 2) evaluated by the estimation of Thiobarbituric Acid Reactive Substances (TBARS) levels in the hippocampus homogenate compared with the control group (P<0.001). The co-administration of P. graveolens EO significantly alleviated LPO levels in the hippocampus tissue compared with the MET group (P<0.01) 28 days after exposure to MET.
.png)
3.3.2. Nonenzymatic antioxidant reduced GSH levels
Exposure to MET caused a significant reduction in GSH levels in the hippocampus homogenate of the MET-treated group in comparison with the control group (P<0.01) (Figure 2). The coadministration of P. graveolens EO significantly modulated GSH levels (P<0.05) in comparison with the MET group.
3.3.3. Enzymatic antioxidants
As shown in Figure 3, treatment with MET caused a significant decrease in CAT and GST activities in comparison with control rats (P<0.001 and P<0.001, respectively) associated with a significant increase in GPx activity compared with the control groups (P<0.001). Cotreatment with P. graveolens EO of the MET-treated group significantly modulated the CAT, GST, and GPx activities compared with the MET group (P<0.05, P<0.01, P<0.01, respectively). The P. graveolens restored the antioxidant enzyme activities in the hippocampus.
.png)
3.5 Histopathological findings
The hippocampal area was stained with cresyl violet (Nissl) to evaluate the morphology of the CA1 hippocampal neurons. In the present study, control and P. graveolens EO rats showed a normal neuronal view and cells arranged in alignment with visible cytoplasm and a nucleus with prominent nucleoli (Figures 4 and 5). However, the neurons in the CA1 hippocampal region of MET-treated rats were extensively affected and appeared shrunken with dark cytoplasm and pyknotic nuclei (Figure 6). The coadministration of P. graveolens EO reduced the histopathological appearance and the number of affected cells was less than that in the MET group (Figure 7).
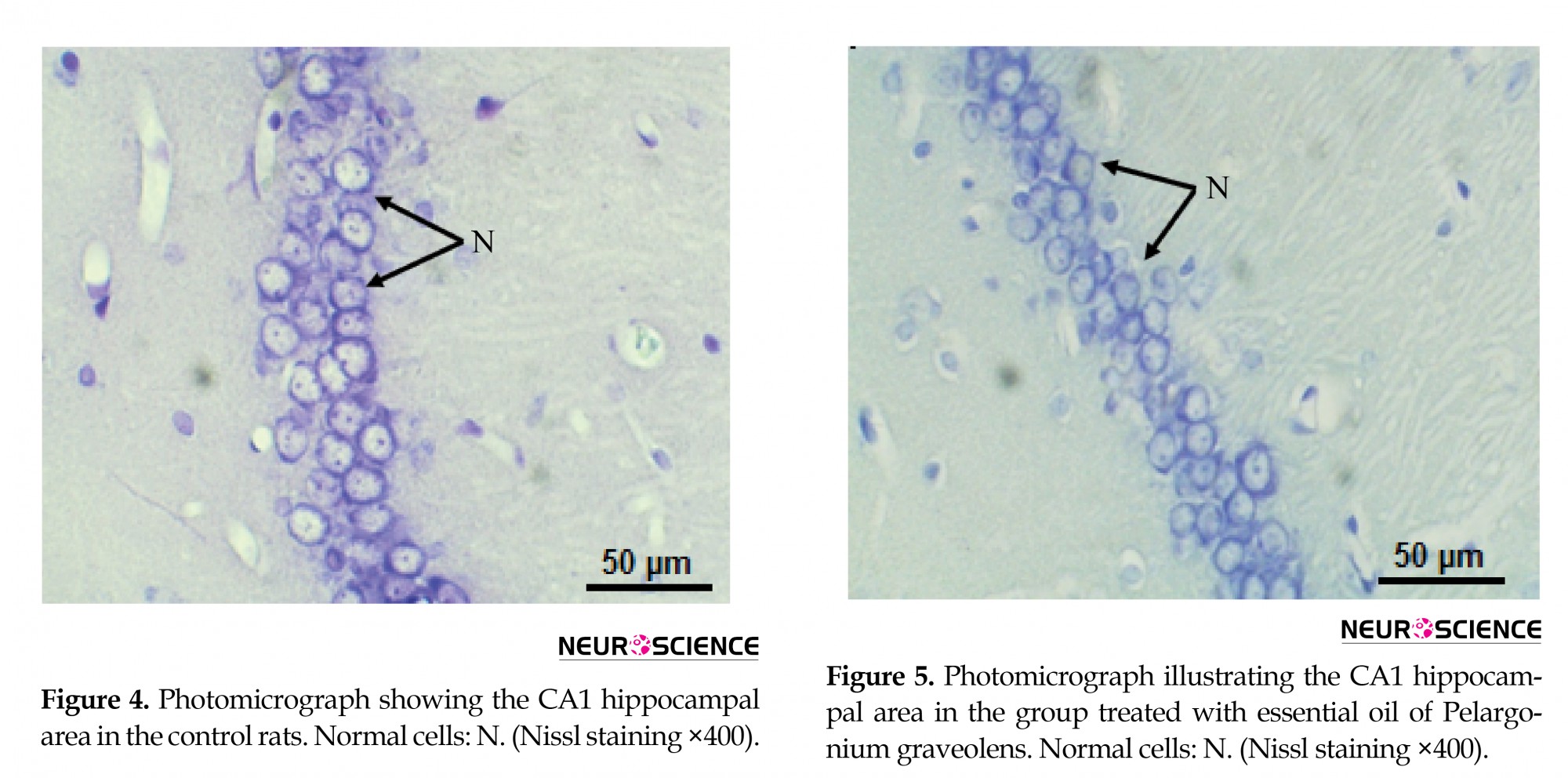
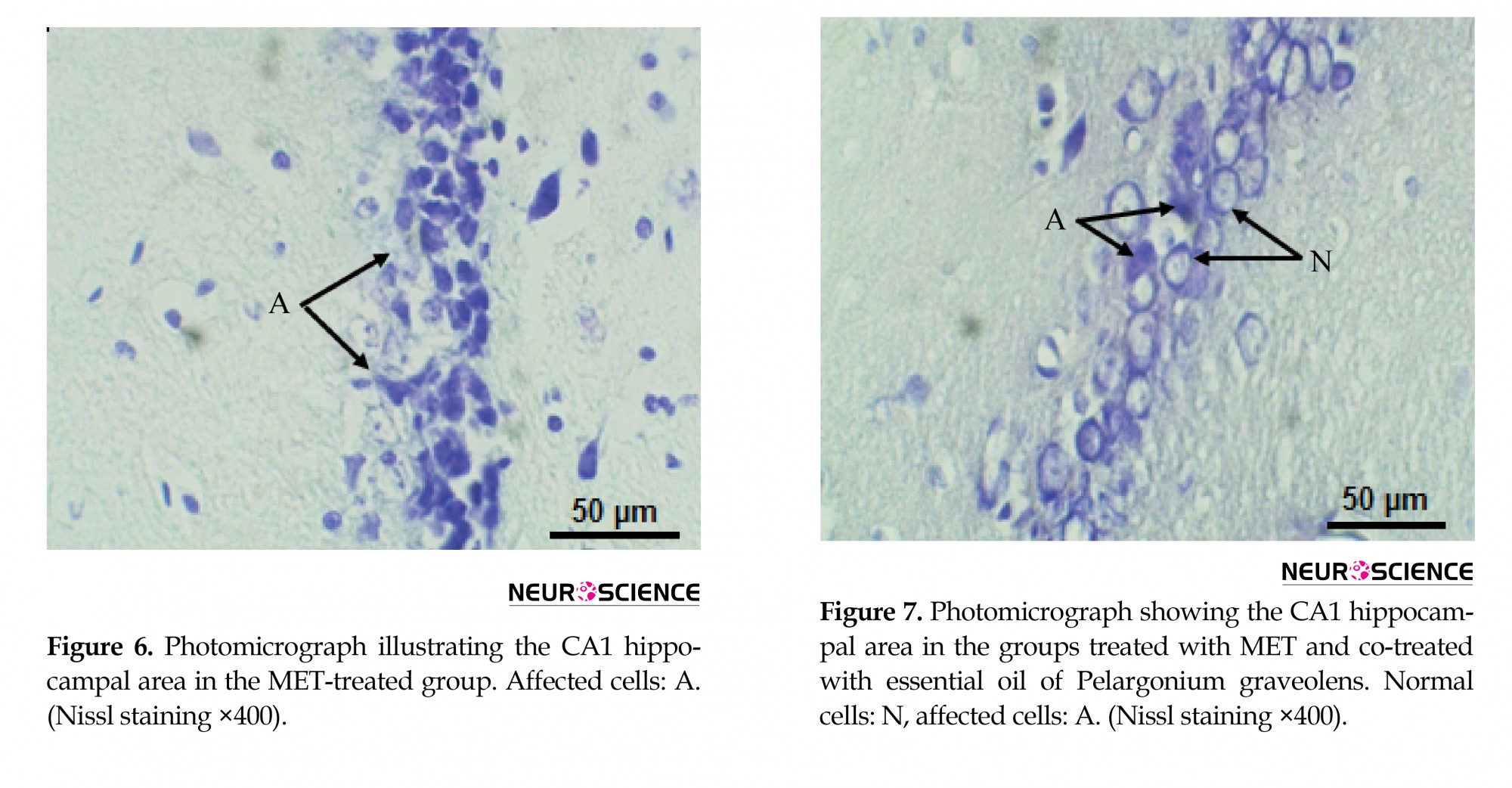
4. Discussion
MET is a carbamate insecticide widely used in agriculture to control a wide range of insects. Besides its advantages in agriculture, it is known to cause a serious negative impact on the environment and health. This study aimed at investigating the neurotoxic effects of MET on the hippocampus of adult male Wistar rats 28 days after treatment with P. graveolens.
The findings of the present study indicated that exposure to MET impaired SWM of the rats. We noted a significant decrease in the percentage of correct alternations in the MET-treated group compared with the controls. The deficits in working memory performance may be attributed to alterations in synaptic plasticity in response to MET exposure (data not shown). These observations of memory deficits were similar to the study published by Chen et al. (2014) who reported that exposure to pesticide altered memory by disturbance plasticity and synaptic transmission in the hippocampus. Exposure to cholinesterase-inhibiting pesticides negatively affects memory and cognition (Chen et al., 2012; Kapil et al., 2010). Other studies have demonstrated that memory disorders after exposure to pesticides is associated with morphological changes and oxidative damage in neurons of the hippocampus (Gasmi et al., 2017; Brocardo, Pandolfo, Takahashi, Rodrigues, & Dafre, 2005).
In our investigation, the co-administration of P. graveolens EO improved SWM in the MET-treated group. The beneficial effects of P. graveolens EO could be attributed to the presence of chemical components engineered to reduce memory loss and improve cognitive function. This result proves that P. graveolens EO plays an important role in the amelioration of SWM. Many studies have shown that herbal essential oils can profoundly improve memory performance (Ballard, O’Brien, Reichelt, & Perry, 2002; Loizzo, Ben Jemia, Senatore, Bruno, Menichini, & Tundis, 2013; Lobo, Patil, Phatak, Chandra, 2010). The rate of decline in SWM after MET exposure was not accompanied by significant changes in the number of total arm entries, reflecting no changes in exploratory activity. This result suggests that exploratory behavior and general locomotor activity of the animals were not affected by MET treatment.
Our results showed that MET exposure caused a significant increase in the levels of AST, ALT, and ALP activities in the hippocampus. These results are in agreement with previous studies reporting an increase in the activities of serum AST, ALT, ALP after MET exposure (Karami-Mohajeri & Abdollahi, 2011; El-Demerdash, Attia, & Elmazoudy, 2012; Djeffal, Messarah, Boumendjel, Kadeche, & Feki, 2015). These enzymes are vital for the animal tissues, their activities are linked to the maintenance of amino acid homeostasis, and disturbance of their activities might be an indicator of mitochondrial damage and loss of the functional integrity of the cell membranes (Dahamna, Belguet, Bouamra, Guendouz, & Harzallah, 2011; Cattani et al., 2014). The elevated activities of AST, ALT, and ALP represent hippocampal damage and were accompanied by neuroinflammation and remarkablecell necrosis (Cattani et al., 2014). This drastic increase in transaminases and ALP levels is in accordance with our histopathological findings that revealed severe necrosis in the hippocampus tissue.
Moreover, the results of our investigation also revealed a decrease in the AChE activity of the MET-treated group compared with the control animals. The inhibition of AChE enzyme activity is considered as a significant marker of exposure to anticholinesterase insecticides, such as carbamate and organophosphorus (Varó, Navarro, Amat, & Guilhermino, 2003). Studies have documented that carbamate pesticide can reversibly inhibit acetylcholinesterase to bind the active sites of the enzyme in competition with acetylcholine (Darvesh et al., 2008). The activity of AChE was inhibited by the free radical formation and the disturbed prooxidant/antioxidant balance in the hippocampus tissue, which can be one of the reasons for decreased AChE activity (Jazayeri, Amanlou, Ghanadian, Pasalar, & Amanlou, 2014; Tsakiris, Angelogianni, Schulpis, & Stavridis, 2000). On the other hand, disturbance in a cholinergic system associated with cognitive disorders often affects learning and memory (Bushnell, Levin, & Overstreet, 1995; Chen et al., 2012). However, supplementation of P. graveolens EO to MET-treated animals showed a significant increase in AChE activity compared with the rats treated with MET alone. Our results indicated that P. graveolens EO ameliorated the activities of enzymes induced by MET insecticide in the hippocampus tissues.
Insecticides are toxic compounds released intentionally or accidentally into our environment, including carbamates. The exposure to these toxic substances accelerates oxidative stress in different types of animal tissues, leading to the production of free radicals and alteration of antioxidant enzymes, which might lead to animal tissues disorders (Jain, Banerjee, Ahmed, Arora, & Mediratta, 2013; Mansour, Mossa, & Heikal, 2009).
This study showed a significant increase in LPO level in MET-treated rats, evidenced by a significant increase in TBARS content in the hippocampus tissue. Exposure to insecticide poisoning generally generates free radicals and causes LPO. LPO is mediated through the free radical metabolites and affects the antioxidants and cellular redox status in tissues. LPO is known as one of the major toxic products generated from lipid peroxides during pesticide exposure. The overproduction of LPO can impair cell membrane integrity, which can lead to a decrease in membrane fluidity and resistance resulting in subnormal membrane function (Ince et al., 2017). Moreover, our study revealed a significant decrease in GSH levels in the hippocampus after exposure to MET. GSH is the most important natural antioxidant and plays a crucial role in protecting cells, which prevents free radical damage and helps detoxification by removing harmful chemicals. MET is principally detoxified by conjugating with GSH and metabolized to mercapturic acid that is ultimately excreted in urine (Grzywa et al., 2016). GSH is consumed by GSH-related enzymes under oxidative stress for detoxifying the peroxides produced due to increased LPO (El-Demerdash, Attia, & Elmazoudy, 2012; Asumi, Tonosaki, & Smelling, 2007).
Furthermore, our results indicated a significant decline in the activities of CAT, GPx, and GST in the hippocampus after MET exposure. This disturbance in antioxidant enzymes activities may be a consequence of overproduction in intracellular ROS and /or the depletion of the antioxidant systems due to the involvement of GSH and these enzymes in the detoxification and scavenging of excess ROS induced by MET in the hippocampus. Djeffal et al. (2015) reported that the increased oxidative stress and cellular damage induced by MET treatment were evident by decreased GPx, CAT, and GST levels in the brain, liver, and kidney. The brain due to its higher levels of oxygen consumption is the most susceptible organ to oxidative stress resulting in a redox imbalance that can cause oxidative injury of the hippocampal neurons (Mehta, Parashar, & Udayabanu, 2017). However, the coadministration of P. graveolens EO protects hippocampal tissue by enhancing the cellular antioxidant properties and consequently decreasing the formation of LPO. Our findings revealed that MET induced oxidative stress in the hippocampus that can be successfully treated with the P. graveolens through its antioxidant properties. Previous studies have reported that P. graveolens prevented animal tissue oxidative damage, restored the oxidant/antioxidant balance, and maintained a reducing intracellular environment (Ben Slima, Ali, & Barkallah, 2013; Boukhris, et al., 2012).
In the present study, the histopathological examination of the CA1 region neurons of the control group showed a normal appearance, with neurons having nuclei prominent with visible cytoplasm and clearly delineated. However, sections of the CA1 region of the MET-treated group showed disturbances in the histoarchitecture of the CA1 region and revealed affected neurons that appeared shrunken with dark cytoplasm and pyknotic nuclei. Pesticides with multiple mechanisms of action in the animal tissues often induce cell death through severe necrosis (Parihar et al., 2013, Nasuti et al., 2014; Tan et al., 2009). In the present study, the oxidative damage in the hippocampus after treatment with MET might be well correlated with the histopathological changes in this tissue. However, coadministration of P. graveolens EO in the MET-treated rats showed markedly normal-appearing neurons in the hippocampal CA1 region, except for pyknosis in some neurons. The administration of P. graveolens EO protects the cellular architecture of the hippocampus against oxidative damage through the reduction of protein oxidation and mitochondrial damage induced by MET toxicity.
In conclusion, exposure to MET for 28 days induced oxidative stress and histopathological changes in the hippocampus tissue, leading to a decline in WSM performance. EO of P. graveolens due to its effective antioxidant properties can protect the hippocampus against the adverse effects caused by MET through reduction of oxidative damage and memory improvement.
Ethical Considerations
Compliance with ethical guidelines
All experimental procedures were conducted in accordance with the institutional guidelines for animal care,. The protocol was approved by the Committee for Animal Experimentation of the Higher School Kouba (ref. ENS -K 06/2014).
Funding
This research did not receive any specific grant from funding agencies in the public, commercial, or not-for-profit sectors.
Authors contributions
All authors contributed equally in preparing all parts of the research.
Conflict of interest
The authors declared no conflict of interest.
References
Abdollahi, M., Ranjbar, A., Shadnia, S., Nikfar, S., Rezaie, A. (2004). Pesticides and oxidative stress. A review. Medical Science Monitor, 10(6), 141-7. https://pubmed.ncbi.nlm.nih.gov/15173684/
Aebi, H. (1984). Catalase in vitro. Methods in Enzymology, 105, 121-6. [DOI:10.1016/S0076-6879(84)05016-3]
Andersen, H. R., Debes, F., Wohlfahrt-Veje C., & Murata, K., Grandjean, P. (2015). Occupational pesticide exposure in early pregnancy associated with sex-specific neurobehavioral deficits in the children at school age. Neurotoxicology and Teratology, 47, 1-9. [DOI:10.1016/j.ntt.2014.10.006] [PMID]
Atsumi, T., Tonosaki, K., Smelling, l. (2007). Increases free radical scavenging activity and decreases cortisol level in saliva. Psychiatry Research, 150(1), 89-96. [DOI:10.1016/j.psychres.2005.12.012] [PMID]
Ballard C. G., O’Brien J. T., Reichelt, K., Perry E. K. (2002). Aromatherapy as a safe and effective treatment for the management of agitation in severe dementia: the results of a double-blind, placebo-controlled trial with Melissa. The Journal of Clinical Psychiatry, 63, 553-8. [DOI:10.4088/JCP.v63n0703] [PMID]
Ben Slima, A., Ali, M. B., Barkallah, M. (2013). Antioxidant properties of Pelargonium graveolensL’Her.Essential oil on the reproductive damage induced by deltamethrin in mice as compared to alphatocopherol. Lipids in Health and Disease, 12, 30. [DOI:10.1186/1476-511X-12-30] [PMID] [PMCID]
Bergmeyer, H. U., Scheibe, P., Wahlefeld, A. W. (1978). Optimisation of methods for aspartate aminotransferase and alanine aminotransferase. Clinical Chemistry, 24(1), 58-73. [DOI:10.1093/clinchem/24.1.58] [PMID]
Boukhris, M., Bouaziz, M., Feki, I., J.H., El Feki, A., & Sayadi, S. (2012). Hypoglycemic and antioxidant effects of leaf essential oil of Pelargonium graveolensL’Hér. In alloxan induced diabetic rats. Lipids in Health and Disease, 11, 81. [DOI:10.1186/1476-511X-11-81] [PMID] [PMCID]
Bowers, G. N., McComb, R. B. (1975). Measurement of total alkaline phosphates activity in humain serum. Clinical Chemistry, 21(13), 1988-95. [DOI:10.1093/clinchem/21.13.1988] [PMID]
Bradford, M. M. (1976). A rapid and sensitive method for the quantification of microgram quantities of protein utilising the principle of protein-dye binding. Analytical Biochemistry, 72(1-2), 248-54. [DOI:10.1016/0003-2697(76)90527-3]
Brocardo, P. S., Pandolfo, P., Takahashi, R. N., Rodrigues, A., & Dafre, A. L. (2005). Antioxidant defenses and lipid peroxidation in the cerebral cortex and hippocampus following acute exposure to malathion and/or zinc chloride. Toxicology, 207(2), 283-91. [DOI:10.1016/j.tox.2004.09.012] [PMID]
Buege, J. A., & Aust, S. D. (1984). Microsomal lipid peroxidation. Methods in Enzymology, 105, 302-10. [DOI:10.1016/S0076-6879(78)52032-6]
Bushnell, P. J., Levin, E. D., & Overstreet, D. H. (1995). Spatial working and reference memory in rats bred for autonomic sensitivity to cholinergic stimulation: Acquisition, accuracy, speed and effects of cholinergic drugs. Neurobiology of Learning and Memory, 63(2), 116-32. [DOI:10.1006/nlme.1995.1012] [PMID]
Cattani, D., Liz, O., Cavalli, V. L., Heinz, R. C., Domingues, J. T., & Dal-Cim, T., et al. (2014). Mechanisms underlying the neurotoxicity induced by glyphosate-based herbicide in immature rat hippocampus: Involvement of glutamate excitotoxicity. Toxicology, 320(5), 34-45. [DOI:10.1016/j.tox.2014.03.001] [PMID]
Chen, N. N., Luo, D. J., Yao, X. Q., Yu, C., Wang, Y., & Wang, Q. et al. (2012). Pesticides induce spatial memory deficits with synaptic impairments and an imbalanced tauphosphorylation in rats. Journal of Alzheimer's Disease, 30(3), 585-94. [DOI:10.3233/JAD-2012-111946] [PMID]
Dahamna, S., A. Belguet, D., Bouamra, A., Guendouz, M., & Harzallah, D. (2011). Evaluation of the toxicity of cypermethrin pesticide on organs weight loss and some biochemical and histological parameters. Communications in Agricultural and Applied Biological Sciences, 76(4), 915-21. https://pubmed.ncbi.nlm.nih.gov/22702208/
Deacon, R. M., & Rawlins, J. N. (2006).T-maze alternation in the rodent. Nature Protocols, 1(1), 7-12. [DOI:10.1038/nprot.2006.2] [PMID]
Djeffal, A., Messarah, M., Boumendjel, A., Kadeche, L., & Feki, A. E. (2015). Protective effects of vitamin C and selenium supplementation on methomyl-induced tissue oxidative stress in adult rats. Toxicology and Industrial Health, 31(1), 31-43. [DOI:10.1177/0748233712468020] [PMID]
Doherty, J. D. (2006). Screening pesticides for neuropathogenicity. BioMed Research International, 2006(3), 70414. [DOI:10.1155/JBB/2006/70414] [PMID] [PMCID]
Darvesh, S., Darvesh, K. V., McDonald, R. S., Mataija, D., Walsh, R., & Mothana, S., et al. (2008). Carbamates with differential mechanism of inhibition toward acetylchoilnesterase and butyrylcholinesterase. Journal of Medicinal Chemistry, 51, 4200-12. [DOI:10.1021/jm8002075] [PMID]
El-Demerdash, F. M. (2011). Lipid peroxidation, oxidative stress and acetylcholinesterase in rat brain exposed to organophosphate and pyrethroid insecticides. Food and Chemical Toxicology, 49(6), 1346-52. [DOI:10.1016/j.fct.2011.03.018] [PMID]
El-Demerdash, F., Attia, A. A., & Elmazoudy, R. H. (2012). Biochemical and histopathological changes induced by different time intervals of methomyl treatment in mice liver. Journal of Environmental Science and Health, Part A, 47(12), 1948-54. [DOI:10.1080/03601234.2012.676513] [PMID]
Ellman, G. L. (1959). Tissue sulfhydryl groups. Archives of Biochemistry and Biophysics, 82(1), 70-7. [DOI:10.1016/0003-9861(59)90090-6]
Flohe, L., Gunzler, W.A. (1984). Analysis of glutathione peroxidase. Methods in Enzymology, 105, 114-21. [DOI:10.1016/S0076-6879(84)05015-1]
Gasmi, S., Rouabhi, R., Kebieche, M., Boussekine, S., & Salmi, A., et al. (2017). Effects of Deltamethrin on striatum and hippocampus mitochondrial integrity and the protective role of Quercetin in rats. Environmental Science and Pollution, 24(19), 16440-57. [DOI:10.1007/s11356-017-9218-8] [PMID]
Grzywa, R., Winiarski, Ł., Psurski, M., Rudnicka, A., Wietrzyk, J., & Gajda, T., et al. (2016). Synthesis and biological activity of diisothiocyanate-derived mercapturic acids. Bioorganic & Medicinal Chemistry Letters, 26, 667-71. [DOI:10.1016/j.bmcl.2015.11.045] [PMID]
Habig, W. H., Pabst, M. J., & Jakoby, W. B. (1974). Glutathione- S-transferase the first step in mercapturic acid formation. Journal of Biological Chemistry, 249(22), 7130-9. https://www.jbc.org/content/249/22/7130.full.pdf
Ince, S., Arslan-Acaroz, D., Demirel, H. H., Varol, N., Ozyurek, H.A., & Zemheri, F., et al. (2017). Taurine alleviates malathion induced lipid peroxidation, oxidative stress, and proinflammatory cytokine gene expressions in rats. Biomedicine & Pharmacotherapy, 96, 263-8. [DOI:10.1016/j.biopha.2017.09.141] [PMID]
Jain, S., Banerjee, B. D., Ahmed, R. S., Arora, V. K., & Mediratta, P. K. (2013). Possible role of oxidative stress and brain derived neurotrophic factor in triazophos induced cognitive impairment in rats. Neurochemical Research, 38(10), 2136-47. [DOI:10.1007/s11064-013-1122-0] [PMID]
Jazayeri, B., Amanlou, A., Ghanadian, N., Pasalar, P., & Amanlou, M. (2014). A preliminary investigation of anticholinesterase activity of some Iranian medicinal plants commonly used in traditional medicine. DARU Journal of Pharmaceutical Sciences, 22(1), 17. [DOI:10.1186/2008-2231-22-17] [PMID] [PMCID]
Jollow, D. J., Mitchell, J. R., Zampaglione, N., & Gillette, J. R. (1974). Bromobenzene-induced liver necrosis.Protective role of glutathione and evidence for 3, 4-bromobenzene oxide as the hepatotoxic metabolite. Pharmacology, 11(3), 151-69. [DOI:10.1159/000136485] [PMID]
Kang, H.Y., Na, S.S., & Kim, Y.K. (2010). Effects of oral care with essential oil on improvement in oral health status of hospice patients. Journal of Korean Academy of Nursing, 40(4), 473-81. [DOI:10.4040/jkan.2010.40.4.473] [PMID]
Kapil, D. M., Gobind, R. G., Ashish, K. M., Tarun, A., Amit, K. S., & Naresh, K., et al. (2010).Reversal of propoxur-induced impairment of memory and oxidative stress by 4′-chlorodiazepam in rats. Naunyn-Schmiedeberg’s Archives of Pharmacology, 381(1), 1-10. [DOI:10.1007/s00210-009-0475-z] [PMID]
Karami-Mohajeri S., & Abdollahi, M. (2011). Toxic influence of organophosphate, carbamate, and organochlorine pesticides on cellular metabolism of lipids, proteins, and carbohydrates: A systematic review. Human and Experimental Toxicology, 30(9), 1119-40. [DOI:10.1177/0960327110388959] [PMID]
Karczmar, A. (1998). Anticholinesterases dramatic aspects of their use and misuse. Neurochemistry International, 32(5-6), 401-11. [DOI:10.1016/S0197-0186(97)00123-X]
Levin, E. D., Addy, N., Baruah, A., Elias, A., Cannelle, C. N., Seidler, F. J. et al. (2002). Prenatal chlorpyrifos exposure in rats causes persistent behavioral alterations. Neurotoxicology and Teratology, 24(6), 733-41. [DOI:10.1016/S0892-0362(02)00272-6]
Lobo, V., Patil, A., Phatak, A., & Chandra, N. (2010). Free radicals, antioxidants and functional foods: Impact on human health. Pharmacognosy Reviews, 4(8), 118-26. [DOI:10.4103/0973-7847.70902] [PMID] [PMCID]
Loizzo M. R., Ben Jemia M., Senatore F., Bruno M., Menichini F., & Tundis R. (2013). Chemistry and functional properties in prevention of neurodegenerative disorders of five Cistus species essential oils. Food Chemistry Toxicology, 59, 586-94. [DOI:10.1016/j.fct.2013.06.040] [PMID]
Manjeet, S., & Charles, R. (2012). Environmental exposure to pesticides and neurodegenerative diseases. Journal of Alzheimer Disease & Parkinsonism, 2, 3. [DOI:10.4172/2161-0460.1000e116]
Mansour, S. A., Mossa, A. H., & Heikal, T. M. (2009). Effect of methomyl on lipid peroxidation and antioxidant enzymes in rat erythrocytes: In vitro studies. Toxicology and Industrial Health, 25(8), 557-63. [DOI:10.1177/0748233709349829] [PMID]
Mehta, V., Parashar, A., & Udayabanu, M. (2017). Quercetin prevents chronic unpredictable stress induced behavioral dysfunction in mice by alleviating hippocampal oxidative and inflammatory stress. Physiology & Behavior, 171, 69-78. [DOI:10.1016/j.physbeh.2017.01.006] [PMID]
Murat, K., Onder, Y., Caner, F. D., Hasan, H. O., Ibrahim, B., & Salih, C., et al. (2015). Insecticide imidacloprid influences cognitive functions and alters learning performance and related gene expression in a rat model. International Journal of Experimental Pathology, 96 (5), 332-7. [DOI:10.1111/iep.12139] [PMID] [PMCID]
Nasuti, C., Fattoretti, P., Carloni, M., Fedeli, D., Ubaldi, M., & Ciccocioppo, R., et al. (2014). Neonatal exposure to permethrin pesticide causes lifelong fear and spatial learning deficits and alters hippocampal morphology of synapses. Journal of Neurodevelopmental Disorders, 6(1), 7-18. [DOI:10.1186/1866-1955-6-7] [PMID] [PMCID]
Nieradko-Iwanicka, B., & Borzęcki, A. (2015). Subacute poisoning of mice with deltamethrin produces memory impairment, reduced locomotor activity, liver damage and changes in blood morphology in the mechanism of oxidative stress. Search Results Pharmacological Reports, 67(3), 535-41. [DOI:10.5604/01.3001.0010.3827] [PMID]
Olga, A., Timofeeva, C. S., Roeggeb, F. J., Seidlerc, T. A., & Slotkinac, E. D. (2008). Persistent cognitive alterations in rats after early postnatal exposure to low doses of the organophosphate pesticide, diazinon. Neurotoxicology and Teratology, 30(1), 38-45. [DOI:10.1016/j.ntt.2007.10.002] [PMID] [PMCID]
Parihar, V. K., Hattiangady, B., Shuai, B., & Shetty, A. K. (2013). Mood and memory deficits in a model of Gulf War illness are linked with reduced neurogenesis, partial neuron loss, and mild inflammation in the hippocampus. Neuropsychopharmacology, 38(12), 2348-62. [DOI:10.1038/npp.2013.158] [PMID] [PMCID]
Qing, C., Yujie, N., Rong, Z., Huicai, G., Yanjie, G., & Yao, L., et al. (2010). The toxic influence of paraquat on hippocampus of mice: Involvement of oxidative stress. Neuro Toxicology, 31(3), 310-6. [DOI:10.1016/j.neuro.2010.02.006] [PMID]
Swonger, A. K., & Rech, R. H. J. (1972). Serotonergic and cholinergic involvement in habituation of activity and spontaneous alternation of rats in a Y maze. Journal of Comparative and Physiological Psychology, 81(3),509-22. ISSN: 0021-9940. [DOI:10.1037/h0033690] [PMID]
Tan, D. H., Peng, S. Q., Wu, Y. L., Wang, Y. M., Lu, C. F., & Ding, W., et al. (2009). Chlorpyrifos induces delayed cytotoxicity after withdrawal in primary hippocampal neurons through extracellular signal-regulated kinase inhibition. Biological and Pharmaceutical Bulletin, 32(10), 1649-55. [DOI:10.1248/bpb.32.1649] [PMID]
Terry, A. V. Jr., Stone, J. D., Buccafusco, J. J., Sickles, D. W., Sood, A., & Prendergast, M. A. (2003). Repeated exposures to subthreshold doses of chlorpyrifos in rats.Hippocampal damage, impaired axonal transport, and deficits in spatial learning. Journal of Pharmacology and Experimental Therapeutics, 305(1), 375-84. [DOI:10.1124/jpet]
Tsakiris, S., Angelogianni, P., Schulpis, K. H., & Stavridis, C. (2000). Protective effect of L-phenylalanine on rat brain acetylcholineesterase inhibition induced by free radicals. Clinical chemistry, 33 (2), 103-6. [DOI:10.1016/S0009-9120(99)00090-9]
Valiollah, H., Alireza, G., & Hadi, J. (2004). Black cumin seed essential oil, as a potent analgesic and antiinflammatory drug. Phytotherapy Research, 18(3), 195-9. [DOI:10.1002/ptr.1390] [PMID]
Varó, I., Navarro, J. C., Amat, F., & Guilhermino, L. (2003). Effect of dicholorvos on cholinesterase activity of the European sea bass (Dicentrarchus labrax). Pesticide Biochemistry and Physiology, 75, 61-72. [DOI:10.1016/S0048-3575(03)00019-1]
Virginia, R., Srikesh, A., Megan, H., Frederica, P., Lori, H., & Dana, B. B., et al. (2011). Seven-year neurodevelopmental scores and prenatal exposure to chlorpyrifos, a common agricultural pesticide. Environmental Health Perspectives, 119(8), 1196-201. [DOI:10.1289/ehp.1003160] [PMID] [PMCID]
World Health Organization. (2004). The WHO recommended classification of pesticides by hazard and guidelines to classification. Geneva, Switzerland: World Health Organization.
Yan, D., Zhang, Y., Liu, L., & Yan, H. (2016). Pesticide exposure and risk of Alzheimer’s disease: A systematic review and meta-analysis. Scientific Reports, 6, 32222. [DOI:10.1038/srep32222] [PMID] [PMCID]